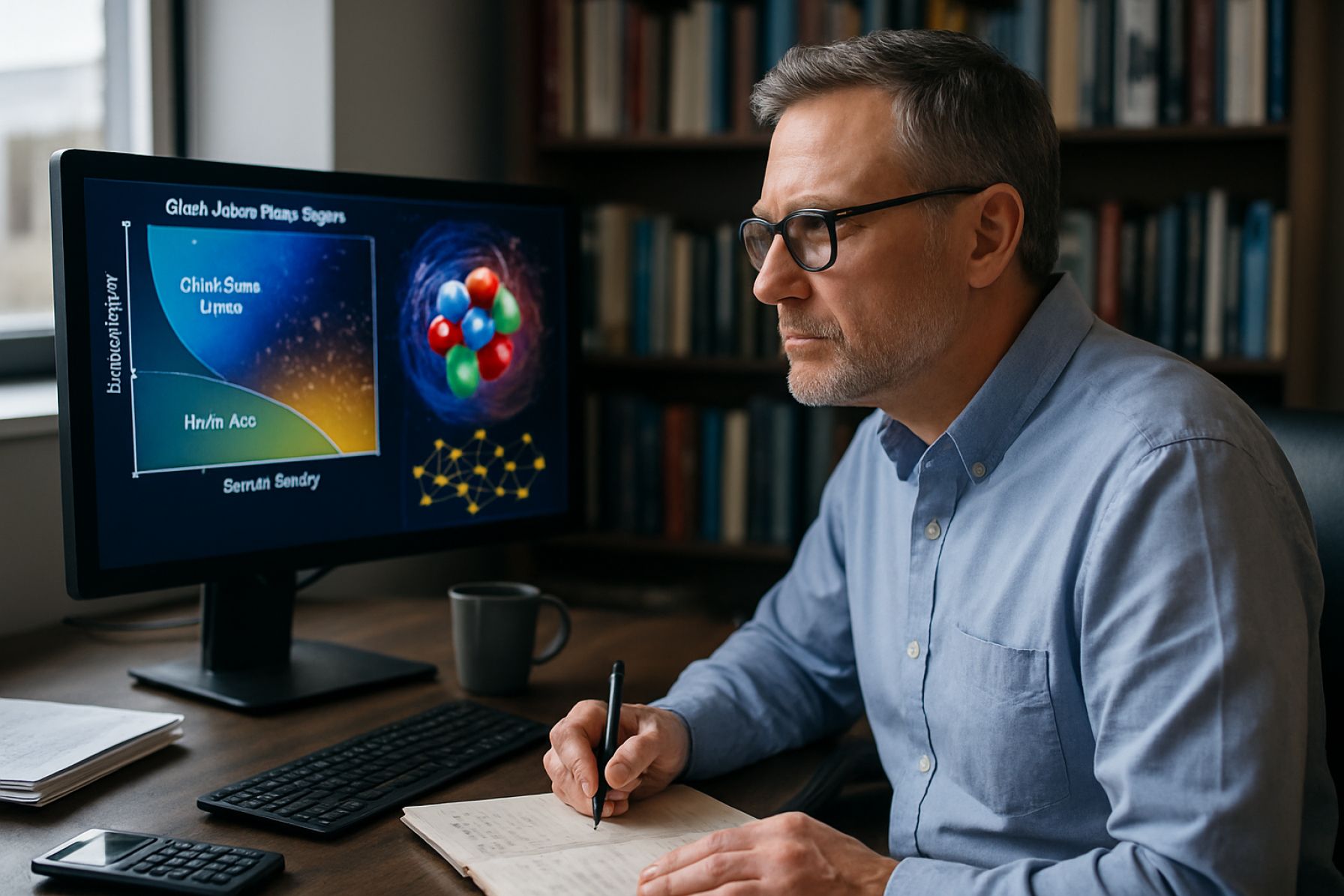
Why 2025 Will Be a Game-Changer for Quark-Hadron Kinetics Modeling—New Technologies, Market Expansion, and the Road to Quantum-Scale Innovation
- Executive Summary: 2025 Outlook for Quark-Hadron Kinetics Modeling
- Key Market Drivers and Inhibitors Shaping the Sector
- Latest Advances in Quark-Hadron Simulation Technologies
- Major Players and Research Institutions: Profiles and Partnerships
- Emerging Applications in Particle Physics, Astrophysics, and Beyond
- Regional Analysis: Hotspots for Investment and Innovation
- Market Forecasts: Growth Projections Through 2030
- Regulatory and Standardization Landscape (e.g., CERN, IEEE)
- Challenges and Unmet Needs in Modeling Accuracy and Scalability
- Future Outlook: Disruptive Trends and Next-Gen Opportunities
- Sources & References
Executive Summary: 2025 Outlook for Quark-Hadron Kinetics Modeling
Quark-hadron kinetics modeling stands at a pivotal juncture in 2025, as advances in computational physics, high-performance computing (HPC), and experimental particle physics converge to refine our understanding of the transition between quark-gluon plasma (QGP) and hadronic matter. This interdisciplinary field underpins research into the early universe, neutron stars, and high-energy heavy-ion collisions, with significant emphasis placed on simulating and interpreting data from large-scale experiments.
The past year has seen substantial progress from major international collaborations leveraging upgraded detector arrays and enhanced computational resources. Facilities such as CERN, with its Large Hadron Collider (LHC), continue to be at the forefront, conducting experiments with unprecedented energy densities and collecting expansive datasets pertinent to QGP formation and evolution (CERN). Furthermore, the Brookhaven National Laboratory, operating the Relativistic Heavy Ion Collider (RHIC), remains a global leader in exploring quark-hadron transition dynamics under varying conditions of temperature and baryon density (Brookhaven National Laboratory).
On the modeling front, specialized software frameworks and open-source codes, such as those developed and maintained by the US Department of Energy’s Exascale Computing Project, are being integrated into multi-physics platforms to simulate the non-equilibrium processes governing the QGP-to-hadron transition (U.S. Department of Energy). These codes increasingly incorporate machine learning algorithms and data-driven approaches, enabling faster parameter scans and better alignment between theory and experimental observables.
2025 is expected to bring further integration of quantum computing prototypes into selected modeling workflows, with collaborations between physics laboratories and technology companies exploring the use of quantum algorithms for simulating strongly coupled quantum chromodynamics (QCD) systems. Initiatives supported by major HPC centers, such as those managed by IBM and Intel, are poised to enhance the fidelity and scalability of kinetic models, particularly as exascale computing becomes more widely accessible.
Looking ahead, the outlook for quark-hadron kinetics modeling is marked by continued cross-institutional collaboration and the prioritization of open data sharing. Ongoing detector upgrades at CERN and RHIC, along with growing partnerships with computational technology providers, are set to enable more granular studies of QGP evolution and hadronization processes. The field is expected to benefit from both incremental algorithmic improvements and disruptive breakthroughs in computational hardware, keeping it at the heart of fundamental physics for the remainder of the decade.
Key Market Drivers and Inhibitors Shaping the Sector
The field of quark-hadron kinetics modeling is poised for significant advancements in 2025 and the following years, shaped by a confluence of scientific, technological, and infrastructural drivers, alongside persistent inhibitors. At the heart of this sector is the quest to understand the transition between quark-gluon plasma (QGP) and hadronic matter, a phenomenon key to both high-energy nuclear physics and cosmology.
One of the primary market drivers is the continued investment in—and data output from—leading particle collider facilities. The ongoing operation and planned upgrades at the CERN Large Hadron Collider (LHC) are central, with experiments like ALICE dedicated to probing QGP and hadronization at unprecedented energy densities. The LHC’s High-Luminosity upgrade, expected to yield higher collision rates through the late 2020s, ensures a steady influx of high-quality data for quark-hadron transition modeling. Similarly, the Brookhaven National Laboratory Relativistic Heavy Ion Collider (RHIC) continues to produce critical experimental results, and its sPHENIX detector, commissioned in 2023, is designed specifically to elucidate QGP properties and the dynamics of hadronization.
Another driver is the expansion of computational resources and collaborative frameworks. The adoption of advanced high-performance computing, such as those provided by the Oak Ridge Leadership Computing Facility, allows for more precise and complex simulations of quark-hadron kinetic processes. Open science initiatives, including data sharing and joint code development between institutions, accelerate theoretical model validation and the refinement of simulation tools.
However, several inhibitors persist. The extreme complexity of quantum chromodynamics (QCD) at the relevant energy scales means that first-principles calculations remain computationally intensive and often require simplifications. This bottleneck limits the pace at which models can be validated against experimental data. Additionally, the lack of standardized software environments and modeling frameworks across research groups hampers reproducibility and collaborative progress. Funding uncertainties, especially for large-scale facilities and international collaborations, may also affect the sector’s growth in the near future.
Looking ahead, the sector’s outlook remains robust, particularly with new experimental facilities on the horizon such as the Electron-Ion Collider (EIC) in the United States. This facility, under development by Brookhaven National Laboratory and Thomas Jefferson National Accelerator Facility, is projected to provide novel insights into the structure and dynamics of nuclear matter, further fueling advances in quark-hadron kinetics modeling. As data volume and computational capabilities rise, the next few years are expected to yield more predictive and experimentally validated models, despite the sector’s technical and organizational challenges.
Latest Advances in Quark-Hadron Simulation Technologies
Quark-hadron kinetics modeling continues to be a focal area in computational nuclear and particle physics, with significant developments expected through 2025 and beyond. The modeling of the transition between quark-gluon plasma (QGP) and hadronic matter—central to understanding early-universe conditions and heavy-ion collisions—relies on increasingly sophisticated simulation frameworks and high-performance computing capabilities.
Recent years have seen the adoption of hybrid models that couple relativistic hydrodynamics (for QGP evolution) to hadronic transport codes, enabling more accurate tracking of the kinetic freeze-out and chemical decoupling processes. Notably, the CERN Large Hadron Collider (LHC) collaborations, such as ALICE, have leveraged these models to interpret data from Pb-Pb collisions, providing new constraints on QGP viscosity and the dynamics of hadronization. These experimental insights have prompted parallel refinement of kinetic models, including the integration of parton cascade modules and improved cross-section parametrizations for hadron interactions.
On the computational side, the deployment of exascale supercomputers at facilities operated by Brookhaven National Laboratory and Oak Ridge National Laboratory is facilitating more detailed and statistically robust event-by-event simulations. These resources underpin the development of open-source codes such as UrQMD, SMASH, and MUSIC, which are widely used in the community for modeling the complex, non-equilibrium evolution of strongly interacting matter. The move towards modular, interoperable simulation frameworks is expected to accelerate, particularly with the aim of bridging the gap between QCD-based microscopic transport and macroscopic hydrodynamic descriptions.
A notable trend for 2025 is the integration of machine learning algorithms to optimize model parameters and rapidly scan the vast multidimensional space of initial conditions and transport coefficients. Several groups collaborating under the U.S. Department of Energy efforts, as well as European initiatives, are investing in surrogate modeling and uncertainty quantification to improve predictive power and facilitate comparison with experimental observables.
Looking forward, the upcoming high-luminosity runs at the LHC and the commissioning of upgrades at the GSI Helmholtz Centre for Heavy Ion Research’s FAIR facility are poised to deliver higher-precision data across a wider range of collision energies. This will provide further impetus for the refinement of quark-hadron kinetics models, particularly in the search for signatures of a critical point in the QCD phase diagram and the study of baryon-rich matter. As experimental and computational capabilities converge, the field anticipates a new era of quantitatively reliable, theory-guided simulation tools for unraveling the complexities of strong interactions.
Major Players and Research Institutions: Profiles and Partnerships
The field of quark-hadron kinetics modeling—central to understanding the strong interaction regime of quantum chromodynamics (QCD)—is driven by a cohort of high-impact research institutions, large-scale collaborations, and select technology companies with advanced computational capabilities. As of 2025, this sector is characterized by a dynamic interplay between experimental facilities, university consortia, national laboratories, and supercomputing centers.
Leading the charge are major international laboratories. CERN remains at the forefront, leveraging the Large Hadron Collider’s (LHC) heavy-ion program and its ALICE experiment to produce high-statistics data on the quark-gluon plasma (QGP) and probe the hadronization process in unprecedented detail. Complementing this, the United States’ Brookhaven National Laboratory (BNL) continues to operate the Relativistic Heavy Ion Collider (RHIC), supporting experiments such as STAR and PHENIX that have yielded vital insights into the kinetics of quark-hadron transitions.
In Asia, RIKEN and Japan Atomic Energy Agency (JAEA) maintain strong theoretical and computational programs, while China’s Institute of High Energy Physics, Chinese Academy of Sciences increasingly participates in global modeling efforts, particularly through collaborations on future collider projects and large-scale lattice QCD computations.
Key European research institutions include GSI Helmholtzzentrum für Schwerionenforschung in Germany, which is developing the Facility for Antiproton and Ion Research (FAIR). FAIR’s upcoming experiments, expected to begin in the next few years, will provide new data for benchmarking and refining kinetic models of strongly interacting matter.
On the computational front, partnerships with supercomputing centers are vital. Oak Ridge National Laboratory (ORNL) and Lawrence Livermore National Laboratory (LLNL) in the US offer petascale and exascale resources, enabling sophisticated simulations of QCD matter and transport phenomena. The European Partnership for Advanced Computing in Europe (PRACE) and Japan’s RIKEN Center for Computational Science also play pivotal roles in supporting large-scale kinetic modeling.
- Collaborative projects: The CERN-led ALICE collaboration, the BNL-based Beam Energy Scan Theory (BEST) initiative, and the FAIR Theory Collaboration exemplify cross-institutional partnerships focused on integrating theory, simulation, and experiment.
- Industry partnerships: While the sector is research-driven, select technology firms such as IBM and NVIDIA provide critical hardware and AI-assisted modeling tools to accelerate quantum transport and hadronization simulations.
Looking ahead, the next few years will see deeper integration between experimental data from new facilities (e.g., FAIR, NICA in Russia), exascale computing, and advanced kinetic frameworks. Expect further convergence between large laboratories, computational research centers, and industry partners as quark-hadron kinetics modeling pushes toward higher precision and new discoveries in QCD matter.
Emerging Applications in Particle Physics, Astrophysics, and Beyond
Quark-hadron kinetics modeling, the computational simulation of the complex transitions between quark-gluon plasma and hadronic matter, stands at the forefront of contemporary particle physics and astrophysics research. Entering 2025, emerging applications are leveraging advances in high-performance computing, quantum simulation, and cross-disciplinary methodologies to probe some of the most fundamental questions in matter evolution and cosmic history.
In high-energy heavy-ion collision experiments, such as those conducted at the CERN Large Hadron Collider (LHC) and the Brookhaven National Laboratory Relativistic Heavy Ion Collider (RHIC), state-of-the-art kinetic models are being used to reconstruct the space-time dynamics of quark-gluon plasma (QGP) formation and hadronization. The upcoming LHC Run 4, scheduled through 2025 and beyond, is expected to yield unprecedented data volumes, challenging theorists to refine and validate their quark-hadron transition models for enhanced predictive power. These models are critical for interpreting signals of deconfinement and chiral symmetry restoration, key phenomena for understanding the early universe and neutron star interiors.
Astrophysics sees parallel advances, with the modeling of quark-hadron transitions central to simulating neutron star mergers and their gravitational wave signatures. Collaborations such as the LIGO Scientific Collaboration are integrating kinetic modeling into multi-messenger astrophysics frameworks, aiming to connect gravitational wave and electromagnetic observations with microphysical processes during extreme astrophysical events.
On the computational front, the integration of machine learning techniques with traditional kinetic theory is rapidly accelerating progress. Several research groups are collaborating with supercomputing centers like Oak Ridge Leadership Computing Facility to optimize algorithms for quantum chromodynamics (QCD) simulations relevant to quark-hadron transitions. These efforts are expected to enhance the accuracy of equation-of-state models and transport coefficients, essential parameters for both terrestrial and astrophysical applications.
Looking ahead, the field anticipates deeper synergy between theoretical developments and experimental discoveries. The 2025–2027 period may see the first robust constraints on the QCD phase diagram at finite baryon density, resulting from next-generation experiments at facilities such as the Joint Institute for Nuclear Research (JINR) in Dubna and the forthcoming Facility for Antiproton and Ion Research (FAIR) in Germany. These results will drive further refinement of kinetic models and open new windows into the properties of dense and hot matter, bridging gaps between particle physics, astrophysics, and cosmology.
Regional Analysis: Hotspots for Investment and Innovation
Quark-hadron kinetics modeling, which underpins the theoretical and computational study of transitions between quark-gluon plasma and hadronic matter, has seen a global surge in research intensity and investment. As of 2025, several regional centers have emerged as leaders in both fundamental science and the development of high-performance modeling platforms, driven by collaborations between universities, national laboratories, and technology suppliers.
In Europe, Germany’s GSI Helmholtzzentrum für Schwerionenforschung and France’s Commissariat à l'énergie atomique et aux énergies alternatives (CEA) are at the forefront, leveraging heavy-ion accelerator facilities to provide unique data for kinetic model validation. The GSI, in particular, is leading the FAIR (Facility for Antiproton and Ion Research) project, which is expected to generate experimental conditions mimicking the early universe’s quark-hadron transition, thereby providing vital benchmarks for modeling efforts through 2025 and beyond. The European Organization for Nuclear Research, CERN, remains pivotal, especially with ongoing upgrades to the Large Hadron Collider (LHC) and its ALICE experiment focusing on heavy-ion collisions. These sites are magnets for international collaboration and are fostering computational innovation through partnerships with European supercomputing centers.
In North America, the US remains a powerhouse, driven by the Brookhaven National Laboratory (BNL) and its Relativistic Heavy Ion Collider (RHIC), alongside the Argonne National Laboratory (ANL) and the Oak Ridge National Laboratory (ORNL). These institutions are not only generating high-fidelity data but also investing in next-generation algorithms for kinetic modeling, including machine learning-enhanced simulation. The upcoming Electron-Ion Collider at BNL, slated for construction through the later 2020s, is a key site for future investment and international collaboration, with modeling groups anticipating new constraints on hadronization dynamics.
In Asia, China’s commitment is exemplified by the Institute of High Energy Physics (IHEP) and the China Spallation Neutron Source, both of which are expanding experimental and computational capacity. Japan, meanwhile, continues to support large-scale theory collaborations at the RIKEN Nishina Center and the High Energy Accelerator Research Organization (KEK), with active investments in modeling infrastructure and international data-sharing.
Looking forward, regional competition and collaboration are expected to intensify, especially as new experimental data from next-generation facilities become available. Governments and agencies are increasing funding for interdisciplinary efforts that combine data science, high-performance computing, and quantum simulation to tackle open questions in quark-hadron kinetics. As such, Europe, North America, and East Asia will remain the primary hotspots for both innovation and investment in this foundational area of particle and nuclear physics through the remainder of the 2020s.
Market Forecasts: Growth Projections Through 2030
The market for Quark-Hadron Kinetics Modeling is expected to experience gradual but notable growth through 2030, reflecting expanding investments in high-energy and nuclear physics research, alongside emerging applications in astrophysics and materials science. As of 2025, the sector remains highly specialized, with demand primarily driven by large-scale scientific collaborations and national laboratories committed to probing the fundamental structure of matter. Institutions such as CERN and Brookhaven National Laboratory (BNL) continue to lead global initiatives, leveraging advanced computational models to interpret results from heavy-ion collisions and particle accelerator experiments.
The growth trajectory of quark-hadron kinetics modeling is closely tied to ongoing and upcoming experimental campaigns. For example, the Relativistic Heavy Ion Collider (RHIC) at BNL is slated for continued operation through at least 2027, with its sPHENIX detector project entering full data-taking mode in 2025. These initiatives demand increasingly sophisticated simulation frameworks, spurring incremental investments in both in-house software development and collaborations with computational physics entities. Similarly, CERN’s Large Hadron Collider (LHC) upgrade cycles—culminating in the High-Luminosity LHC (HL-LHC) by the latter half of this decade—are expected to generate substantial new data requiring advanced modeling approaches.
A significant driver for sector expansion is the anticipated completion and commissioning of new experimental facilities. The Facility for Antiproton and Ion Research (FAIR) in Germany, managed by GSI Helmholtzzentrum für Schwerionenforschung, is scheduled to commence first experiments by 2027. FAIR’s unique capabilities in producing high-density baryonic matter will likely fuel demand for quark-hadron transition modeling at previously inaccessible energy scales. Additionally, the Electron-Ion Collider (EIC) at BNL, which is expected to become operational by the end of the decade, is projected to further diversify and expand the modeling landscape.
From a supplier perspective, the niche nature of this segment means that specialized software vendors—often spin-offs or direct partners of major research organizations—will play a growing role. Companies such as CERN and GSI continue to provide open-source toolkits and simulation packages, fostering a collaborative ecosystem for innovation and interoperability. Looking ahead, the market forecast suggests steady single-digit annual growth in modeling software and services revenue, consistent with the expansion patterns observed in related scientific computing domains.
In summary, while quark-hadron kinetics modeling will remain a specialized discipline, the period through 2030 is poised for sustained growth, underpinned by global investments in next-generation research infrastructure and the escalating complexity of experimental datasets. This outlook relies heavily on continued funding for flagship facilities and the successful translation of computational advances into practical modeling solutions.
Regulatory and Standardization Landscape (e.g., CERN, IEEE)
Quark-hadron kinetics modeling stands at the intersection of theoretical and experimental high-energy physics, with its regulatory and standardization landscape shaped primarily by global research consortia, national laboratories, and renowned standards organizations. As of 2025, the field is witnessing a concerted effort to harmonize modeling approaches, data formats, and computational protocols, reflecting the increasing complexity and international cooperation in particle and nuclear physics experiments.
The CERN organization remains the central authority orchestrating standards for simulation and data sharing related to quark-hadron transitions. Through collaborations such as the ALICE experiment at the Large Hadron Collider (LHC), CERN dictates protocols for event simulation, detector interface standards, and common data models underpinning quark-gluon plasma studies and hadronization processes. CERN’s open data portal and technical standards are referenced globally, setting expectations for transparency and replicability in modeling results.
On the computational side, the IEEE continues to support and maintain standards for high-performance computing (HPC) hardware and software, which are critical for the intensive simulations required in kinetics modeling. The IEEE’s standards for floating-point arithmetic (IEEE 754) and parallel processing interfaces are widely adopted in physics simulations, ensuring that modeling codes yield consistent results across different computing architectures. These standards are crucial for interoperability, especially as research increasingly leverages distributed and cloud-based computation platforms.
National laboratories, such as the Brookhaven National Laboratory (BNL) in the United States and Japan Atomic Energy Agency (JAEA), contribute to the regulatory framework by publishing best practices for the verification and validation of kinetic models. These institutions align with international standards while tailoring guidance to the specific requirements of experiments like the Relativistic Heavy Ion Collider (RHIC) and Japan Proton Accelerator Research Complex (J-PARC), respectively.
In 2025 and the coming years, there is a marked shift towards open-source toolchains and shared benchmarks, with initiatives such as the HEP Software Foundation (HSF) fostering consensus on software validation and documentation requirements. Efforts are underway to integrate machine learning into kinetic modeling, prompting discussions regarding algorithm transparency and reproducibility standards. Anticipated updates from both CERN and the IEEE are expected to formalize interoperability guidelines, data provenance protocols, and ethical considerations as modeling becomes more automated.
Overall, the regulatory and standardization landscape for quark-hadron kinetics modeling is evolving rapidly, with international organizations taking active roles in shaping a robust, interoperable, and transparent scientific ecosystem for the next generation of high-energy physics research.
Challenges and Unmet Needs in Modeling Accuracy and Scalability
Quark-hadron kinetics modeling remains a highly challenging and rapidly evolving area within high-energy physics, especially as the field approaches 2025. Central to the discipline is the need to accurately simulate the transition between quark-gluon plasma (QGP) and hadronic matter—a process that is both computationally intensive and dependent on precise theoretical frameworks. One of the primary challenges is the multiscale nature of the problem, which requires models to bridge quantum chromodynamics (QCD) at the partonic level with collective, macroscopic hadronization phenomena. As experimental results from facilities such as the Relativistic Heavy Ion Collider (Brookhaven National Laboratory) and the Large Hadron Collider (CERN) provide increasingly detailed data, models must evolve to incorporate new observables and higher precision constraints.
A major unmet need is the scalability of current simulation codes. State-of-the-art event generators and transport models—developed by international collaborations like the ALICE Collaboration—are reaching the limits of existing high-performance computing resources. As modeling efforts push toward event-by-event simulations at finer spatial and temporal resolutions, computational demands soar. There is an urgent need for more efficient algorithms, possibly leveraging advances in artificial intelligence and quantum computing, to handle the exascale computing environments being deployed at institutions such as Oak Ridge National Laboratory and Los Alamos National Laboratory.
Furthermore, the complexity of QCD and the lack of first-principles solutions for hadronization force modelers to rely on effective theories and phenomenological parameterizations. This introduces uncertainties that are difficult to quantify and propagate through to experimental observables. Recent efforts, such as those coordinated under the USQCD Collaboration, are focusing on reducing these uncertainties using lattice QCD and improved effective models, but significant gaps remain, especially in regions of high baryon density relevant to upcoming experiments at facilities like GSI Helmholtzzentrum für Schwerionenforschung in Germany.
Looking ahead, the next few years will likely see the integration of real-time data analysis pipelines with kinetic models, as well as the deployment of hybrid approaches that combine traditional Monte Carlo methods with machine learning. However, the field still lacks standardized benchmarks and shared datasets, hindering reproducibility and cross-validation. As international collaborations intensify and new experimental programs such as the Electron-Ion Collider at Brookhaven National Laboratory come online, addressing issues of accuracy, scalability, and uncertainty quantification will remain at the forefront of quark-hadron kinetics modeling efforts through 2025 and beyond.
Future Outlook: Disruptive Trends and Next-Gen Opportunities
Quark-hadron kinetics modeling is positioned at the intersection of quantum chromodynamics (QCD), computational physics, and advanced simulation methodologies. As of 2025, the field is undergoing significant transformation driven by the convergence of high-performance computing (HPC), artificial intelligence (AI), and novel experimental data from global accelerator facilities. The ability to simulate the complex transitions between quark-gluon plasma and hadronic matter with unprecedented fidelity is poised to impact not only fundamental physics but also emerging applications in nuclear technology, astrophysics, and potentially quantum computing.
A primary driver in the next few years will be the integration of exascale computing resources into quark-hadron transition simulations. Facilities such as the Oak Ridge National Laboratory and Los Alamos National Laboratory are leading the deployment of exascale supercomputers, enabling lattice QCD calculations and kinetic transport models to resolve finer details of phase transitions. These advancements are closely coupled with experimental programs at colliders like the Brookhaven National Laboratory (BNL) Relativistic Heavy Ion Collider (RHIC) and the CERN Large Hadron Collider (LHC), where heavy-ion collision data continue to inform and validate theoretical models.
On the software and algorithmic front, the adoption of machine learning techniques to optimize parameter spaces and accelerate Monte Carlo simulations is gaining momentum. Collaborations such as the USQCD Collaboration are actively developing open-source frameworks that leverage AI-driven surrogate models to predict phase diagram features and rare event kinetics. These tools are expected to streamline the interpretation of large datasets from next-generation experiments and facilitate rapid hypothesis testing.
Looking ahead, the field anticipates disruptive breakthroughs from the synergy of quantum computing and QCD modeling. Quantum algorithms developed by teams at IBM and Intel are being evaluated for their potential to tackle the sign problem of finite-density QCD, a major hurdle in simulating real-time quark-hadron dynamics. Industry partnerships with national labs are set to expand as quantum hardware matures, offering new avenues for simulating non-equilibrium processes and exotic matter states that are currently beyond classical computational reach.
In summary, the next few years are expected to see quark-hadron kinetics modeling evolve from primarily academic exploration to a robust, cross-disciplinary platform. This evolution will be propelled by the fusion of experimental data, exascale and quantum computing, and AI-enhanced simulation tools, with substantial contributions from leading laboratories, technology companies, and international collaborations shaping the trajectory of the field.
Sources & References
- CERN
- Brookhaven National Laboratory
- IBM
- Thomas Jefferson National Accelerator Facility
- CERN
- Oak Ridge National Laboratory
- GSI Helmholtz Centre for Heavy Ion Research
- RIKEN
- Japan Atomic Energy Agency
- Institute of High Energy Physics, Chinese Academy of Sciences
- Lawrence Livermore National Laboratory
- Partnership for Advanced Computing in Europe
- RIKEN Center for Computational Science
- NVIDIA
- LIGO Scientific Collaboration
- Joint Institute for Nuclear Research
- GSI Helmholtzzentrum für Schwerionenforschung
- CERN
- Brookhaven National Laboratory
- Oak Ridge National Laboratory
- Institute of High Energy Physics (IHEP)
- IEEE
- Los Alamos National Laboratory
- USQCD Collaboration